Gravitational waves, tiny ripples in spacetime that Einstein believed are too faint ever to be detected, are revolutionizing both our understanding and our view of the cosmos.
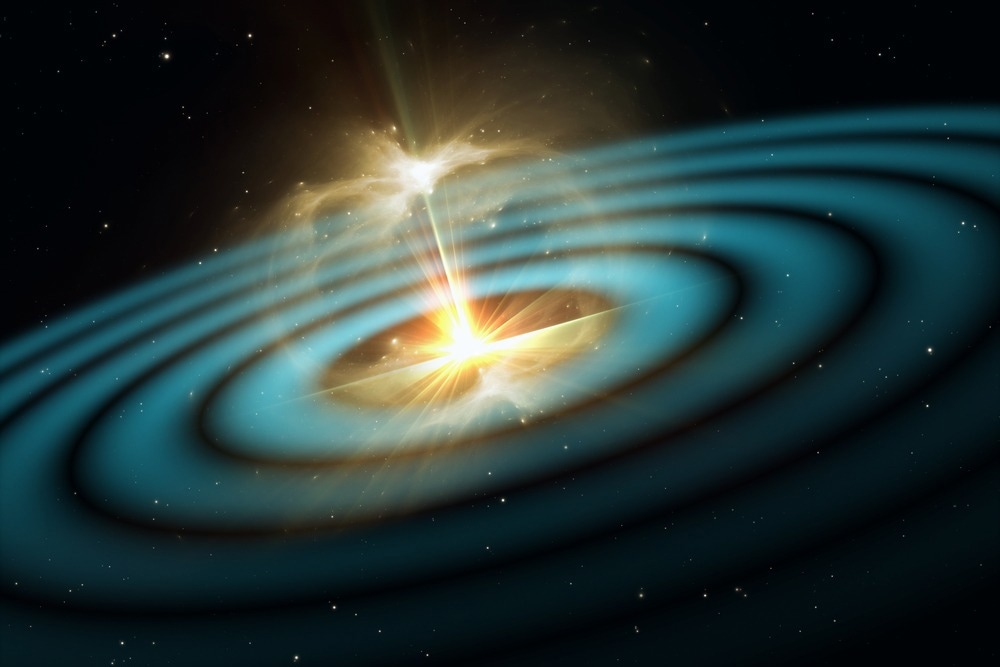
Image Credit: Jurik Peter/Shutterstock.com
Imagine an event occurring in space that is so tremendous and violent that it sets the very fabric of space and time itself ringing like the peels of a struck bell. The ringing of these cosmic bells is so powerful that it can be heard, albeit faintly, over a distance of not miles or even parsecs but over billions of light-years. We call them gravitational waves.
Not only is measuring gravitational waves here on Earth a stunning validation of a 100-plus-year-old theory of gravity, or a feat of engineering that is almost unparalleled, or a window into the most powerful and violent events in the cosmos, but this also offers an entirely new way to “see” the universe.
Gravitational Waves in Theory
Gravitational waves are a concept that arises from Albert Einstein’s magnum opus theory, general relativity, which is currently the best description we have of how gravity operates at truly large scales.
General relativity suggests that objects of mass have a “warping” effect on spacetime, the 4-dimensional unification of space and time that Einstein used to formulate his early theory of special relativity.
This is analogous to taking a rubber sheet and stretching it out, then placing balls of increasing mass upon it. A bowling ball causes more curvature in the sheet than a tennis ball, just as a galaxy causes more curvature in spacetime than a star.
These curves in spacetime influence the passage of matter, so the Earth swirls around the curvature in spacetime caused by the sun, while the moon whips around the “dent” caused by the mass of the Earth, and the sun itself circles the massive curvature in space caused by the supermassive black hole Sagittarius A* at the heart of the Milky Way.
That means that gravity is actually a factor that emerges from the geometry of spacetime. Physicist John Wheeler once infamously said about general relativity, “matter tells spacetime how to curve, and curved spacetime tells matter how to move.”
But objects of mass have more influence on spacetime than this. Einstein also predicted that when objects accelerate — increase speed or change direction — they send ripples propagating through spacetime. A type of gravitational radiation more commonly called gravitational waves.
Circular movement represents constant acceleration, so two objects moving around each other in a circle should send gravitational waves constantly undulating through space. There’s a catch, however. For, say, an accelerating bike rider, the ripples in spacetime that would be caused would be far too faint to ever detect.
But when the accelerating objects have incredible amounts of mass compressed into a tiny radius, such as is the case with a black hole or a neutron star, these gravitational waves would be much stronger.
LIGO says¹ that the strongest gravitational waves emerge from cataclysmic events such as the collision and merger of two black holes, two gravitational waves, or a “mixed merger” between a black hole and a neutron star. Supernovas, triggered when the cores of massive stars collapse at the end of their lives, could also be the source of powerful gravitational wave signals. Another source of these spacetime ripples could be the rotation of neutron stars, some of which can spin as fast as 700 times per second, with tiny bumps on the surface of the stellar remnants giving rise to gravitational waves.
As well as initially predicting their existence, Einstein also made another prediction about gravitational waves. The great scientist believed that these ripples in spacetime, even from the most powerful sources, would be so faint that we could never hope to detect them here on Earth. Fortunately, in this one aspect of gravitational wave science, Einstein was wrong.
Detecting Gravitational Waves Here on Earth
The first hints of gravitational waves outside of theory arrived in 1974, 19 years after the death of Einstein.
Using the Arecibo Radio Observatory in Puerto Rico, astronomers discovered a binary pulsar, two highly magnetized rotating neutron stars that beam out electromagnetic radiation that sweeps over Earth like the light from a cosmic lighthouse. This is exactly the type of powerful extreme system that physicists predict would be a strong radiator of gravitational waves.
As this binary pulsar, located 21,000 light years from Earth, radiates gravitational waves these should carry energy away from it. As a result of this energy loss, the two occupants of the system should be losing angular momentum and thus drawing closer and closer together. Examining how the orbits of these pulsars changed over seven years, astrophysicists Russell Hulse and Joseph Taylor² discovered the two objects were drawing together at exactly the rate that general relativity would predict via the emission of gravitational waves.
Astronomers would continue to study pulsar systems like this, confirming similar orbital “tightening” and further indirectly confirming the existence of gravitational waves. But what physicists really wanted to do was make a direct detection of these ripples in spacetime via the direct interaction between gravitational waves and a piece of equipment.
The problem is creating an experimental set-up sensitive enough to detect gravitational waves that have traveled thousands, millions, or even billions of light years. The answer was the creation of massive laser interferometers³.
Interferometers are devices that were first created in the mid-1800s to measure the speed of light. The most famous use of an interferometer before its application in gravitational wave detectors was as part of the Micelson-Morley experiment, which showed that the aether, a medium through which light was once proposed to propagate, does not exist, arguably the most famous “null result” in the history of science.
Interferometers consist of a laser emitter and a beam splitter that creates two laser arms of equal length. They rely on a phenomenon in quantum physics called “interference.”
When two beams of light are “in phase” the peaks and troughs of their wavelength line up and are amplified in what is called “constructive interference.” When two wavelengths aren’t in phase, peaks meet troughs and cancel each other out causing “destructive interference.” The amplitude of the peaks created is always the sum of the two peaks that created them in interference. So if a peak is a 1 and a trough a -1 you can see how constructive and destructive interference would work.
When the laser is split in the interferometer the separate arms are in phase. That means when they meet up at a detector after being bounced up and down these arms, the beams of light should come back together to show constructive interference.
As gravitational waves travel over the interferometer arms, however, they cause space to squeeze and stretch. That means the distance the two laser beams travel over isn’t the same, and thus the beams are no longer in phase. Thus, when the laser light arrives back at the photodetector, operators would see destructive interference.
This forms the basis of the gravitational wave detectors the Laser Interferometer Gravitational-Wave Observatory (LIGO) in the U.S., Virgo in Italy, and the Kamioka Gravitational Wave Detector (KAGRA) in Japan. The scale of these interferometers is much greater than that of the experiments performed on laboratory tabletops in the 1800s.
For example, each of the two LIGO detectors⁴, based in Livingston, Louisiana, and near Richland, Washington, respectively, is composed of two 2.5 mile (4 kilometer) long laser arms. This size is needed because these observatories need to be sensitive enough to distinguish gravitational waves from more down-to-Earth vibrations, such as those caused by passing traffic.
LIGO’s twin interferometers are designed in such a way that they are so sensitive they can measure a change in distance 1/10,000th the width of a proton!
And finally, on September 14, 2015, almost exactly 100 years after the theory that birthed them was devised, the LIGO team made the first direct detection of a gravitational wave signal. The gravitational wave signal, given the designation GW150914, came from the collision and merger of two black holes located 1.3 billion light years away. Traveling at the speed of light, these ripples in space had been traveling to Earth for an astounding 1.3 billion years, meaning they were launched before humanity even existed.
Since 2015 LIGO, Virgo, and KAGRA have detected a multitude of gravitational waves, some from other black hole mergers, others from the collision of two neutron stars, and even signals from mixed encounters between a black hole and a neutron star. It is the information carried by these signals that is really shaking up astronomy and providing us with a new way of looking at the cosmos.
Mixed Messages: Gravitational Waves and the Future of Astronomy
Gravitational waves carry encoded within them information about the objects and events that launch them through space, meaning the detection of these ripples in spacetime constitutes a new form of astronomy.
Traditional astronomy relies on the detection of electromagnetic radiation, light, over a multitude of frequencies, but gravitational waves or “gravitational radiation” also consist of different frequencies and can traverse objects in space like dense clouds of gas that can absorb certain frequencies of light, undeterred.
Gravitational waves become really powerful, however, when they are combined with observations in electromagnetic radiation. This, along with astronomy that uses near-massless, chargeless “ghost particles” called neutrinos, has created a new window on the universe called “multimessenger astronomy.”⁷
This allows astronomers to catch a gravitational wave signal and then hunt for the source of this signal with traditional astronomy techniques. An impressive validation of multimessenger astronomy came when gravitational waves and photons were detected from the binary neutron star merger GW170817⁸.
And the future of gravitational wave detection itself looks bright. Not only have the Earth-based interferometers LIGO, Virgo, and KAGRA, which form a single collaboration, received sensitivity upgrades for their current and future operating runs. Additionally, the European Space Agency (ESA) and NASA have plans to place a gravitational wave detector in space.
Consisting of three spacecraft separated by millions of miles, the Laser Interferometer Space Antenna (LISA)⁶ will be a gravitational wave detector that is, in principle, larger than Earth.
LISA will be able to hunt for gravitational waves with much lower frequencies than current detectors. These correspond to objects circling each other with much wider orbits. These objects could also be much more massive than those hunted for by LIGO and LISA’s other Earth-based counterparts.
This means LISA could help humanity detect these tiny ripples in spacetime from orbiting black holes millions of times more massive than our sun after its launch, set to occur in the early 2030s.
More from AZoQuantum: The History of Cosmic Ray Research
Sources
¹ What are gravitational waves, LIGO, [accessed 09/05/23], [https://www.ligo.caltech.edu/page/what-are-gw]
² Look deeper, LIGO, [accessed 09/05/23], [https://www.ligo.caltech.edu/page/look-deeper]
³ What is an Interferometer? LIGO, [accessed 09/05/23], [https://www.ligo.caltech.edu/page/what-is-interferometer]
⁴ What is LIGO? LIGO, [accessed 09/05/23], [https://www.ligo.caltech.edu/page/what-is-ligo#:~:text=Each%20LIGO%20detector%20consists%20of,the%20tubes%20from%20the%20environment.]
⁵ GW150914 — THE FIRST DIRECT DETECTION OF GRAVITATIONAL WAVES, LSC, [accessed 09/05/23], [https://www.ligo.org/detections/GW150914.php]
⁶ LISA, NASA, [accessed 09/05/23], [https://lisa.nasa.gov/]
⁷ E. Hamilton., What is multi-messenger astronomy?, UW NEWS, [accessed 09/05/23], [https://news.wisc.edu/what-is-multi-messenger-astronomy/]
⁸ Multi-messenger astrophysics, LIGO MIT, [accessed 09/05/23], [https://ligolab.mit.edu/research/mma]
Disclaimer: The views expressed here are those of the author expressed in their private capacity and do not necessarily represent the views of AZoM.com Limited T/A AZoNetwork the owner and operator of this website. This disclaimer forms part of the Terms and conditions of use of this website.