AZoQuantum spoke to Matthew Walker, associate professor of astrophysics and cosmology at Carnegie Mellon University, about the James Webb Telescope and the dark matter research that hopes to draw on its findings.
Could you please tell us a little about your background and how you came to be involved with the James Webb Space Telescope (JWST)?
I am an observational astronomer, broadly interested in the ‘dark matter’ problem that has confounded astronomers and physicists for almost a century. Most of my work involves using large, ground-based telescopes to measure the chemical composition and motions of stars within the ‘dwarf’ galaxies that orbit as satellites of our own Milky Way.
The dwarf galaxies are the smallest, oldest, and darkest galaxies known – made almost entirely of dark matter. A primary goal is to use the motions of their relatively few stars to infer the amount and spatial distribution of dark matter within these systems. Specifically, I want to know how ‘clumpy’ the dark matter is, since this attribute can distinguish between different theoretical candidates for a dark matter particle. In this way, I hope to use astrophysics to learn about particle physics!
When the first call for proposals to use the James Webb Telescope (JWST) was announced in early 2020, I wondered whether the telescope could finally tackle a project that I have been thinking about for many years, but which has always lain just out of reach, marginally feasible using even the Hubble Space Telescope (HST). Specifically, I want to search dwarf galaxies for ‘wide binary’ star systems, which can survive only if the dark matter is distributed smoothly, without significant clumpiness (more details below).
The problem is that in order to detect wide binaries as pairs of stars at the large distances (about 100,000 light-years) of dwarf galaxies, we require exquisite image quality, with resolution capable of distinguishing two sources separated by only 0.00001 degrees (for reference, this is the angle between the headlights of an oncoming car at a distance of 5000 miles).
Prior to JWST, the only telescope that delivers this quality is the Hubble Space Telescope (HST); however, as a relatively small telescope by modern standards (primary mirror diameter 2.4 m), HST struggles to detect the faintest stars, thereby limiting sample sizes and our ability to find (or rule out) the presence of wide binaries with statistical significance. JWST, on the other hand, will not only deliver images as sharp as Hubble’s, but with its larger (6.5 m diameter) mirror, JWST will see the faint stars that HST cannot.
Last November (2020), I asked my graduate student, Chris Kervick, to calculate, based on what we know about the stellar populations (approximate number, age, chemical composition, and spatial distribution of stars) within each of the known dwarf galaxies, to estimate the numbers of wide binary systems that JWST might be able to detect in each galaxy. To our delight, a handful of dwarf galaxies are sufficiently nearby and rich in stars that we expect JWST to detect tens to hundreds of wide binary systems – if they exist!
We then discovered that one of the most promising dwarf galaxy targets – a system named 'Draco II' after its constellation -– is already approved for observation, as part of JWST’s ‘Early Release Science’ (ERS) program. The ERS projects are designed to do ground-breaking science while also ‘getting to know’ the telescope, using all of its various instruments. The original ERS team was approved to acquire images of Draco II in order to measure the ages of its stars. We wrote a proposal to use those same images to hunt for wide binary stars, arguing that we can add scientific value without consuming additional telescope resources.
A few months later, we were thrilled to receive notification from the Space Telescope Science Institute that our program was approved! So now we are preparing to analyze the data, which we expect to be acquired during the first few months of science observations, in mid-to-late 2022.
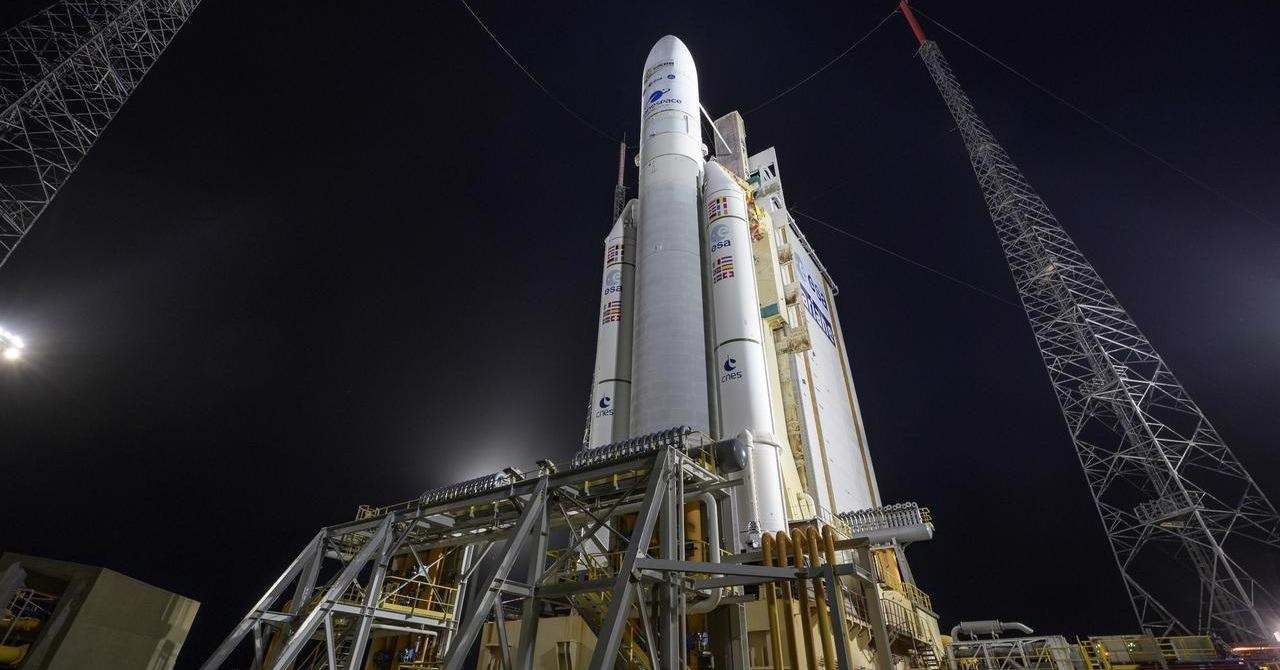
Arianespace's Ariane 5 rocket with NASA’s James Webb Space Telescope onboard, is seen at the launch pad, Thursday, Dec. 23, 2021, at Europe’s Spaceport, the Guiana Space Center in Kourou, French Guiana. The James Webb Space Telescope (sometimes called JWST or Webb) is a large infrared telescope with a 21.3 foot (6.5 meter) primary mirror. The observatory will study every phase of cosmic history—from within our solar system to the most distant observable galaxies in the early universe. Image Credit: (NASA/Bill Ingalls)
What did the development process of this telescope entail? What makes it so extraordinary?
JWST is without question the most ambitious telescope project ever undertaken; it is the hardware embodiment of the phrase ‘high risk, high reward.’ Designs for a ‘next-generation’ space telescope began taking shape soon after the Hubble Space Telescope’s (HST’s) first successes in the mid-1990s.
Driving the design was the ultimate scientific goal of looking far, far away — and thus back in time, given the finite speed at which light propagates — all the way to ‘Cosmic Dawn’, the formation of the very first stars and galaxies. While these early objects emit light primarily at the visible (to the human eye) and ultra-violet wavelengths that HST sees, the expansion of the Universe stretches these wavelengths into the infrared by the time we can observe them today. Therefore, JWST is designed to detect infrared light.
The need for infrared sensitivity presents a multitude of practical challenges and is the reason for JWST’s distinctive appearance. For example, the large solar shield is necessary to keep the telescope and its instruments sufficiently cool that they do not overwhelm the light from distant targets with infrared heat emission from the spacecraft itself.
Another big difference between JWST and HST is that JWST has a much larger primary mirror, 6.5 meters in diameter compared to HST’s 2.4 meters. This means that, all else being equal, JWST gathers seven times more light per observation than HST, making JWST far more sensitive to faint objects.
In fact, JWST’s mirror is so large that a single, continuous piece would have been too bulky to pack for launch; instead, it is an array of 18 hexagonal segments working in tandem. The unfurling and assembly of this array were some of the more nerve-wracking of the early deployment procedures.
Perhaps no aspect of the JWST mission generates more angst than the need to place it in a relatively remote orbit. In order to maintain a constant low (-225 degrees Celsius) temperature for its delicate infrared-sensing instruments, JWST flies in an orbit about the Sun that keeps the Earth between the telescope and the Sun, with small modulations to maintain shade from Sunlight reflected off the Moon. In this orbit, JWST will remain approximately one million miles from Earth, about four times farther away than the Moon.
For comparison, HST circles the Earth, orbiting at an altitude of just 330 miles. HST’s relative proximity has enabled five separate servicing missions, conducted by astronauts aboard the space shuttle (most famously, to correct an aberration in the original primary mirror that had prevented proper focusing). In its distant orbit, JWST cannot be reached for repairs or servicing. This inaccessibility is why astronomers, and especially engineers, are sweating through each of the telescope’s 344 known deployment operations that could end in unrecoverable malfunction. It is truly a high-wire act!
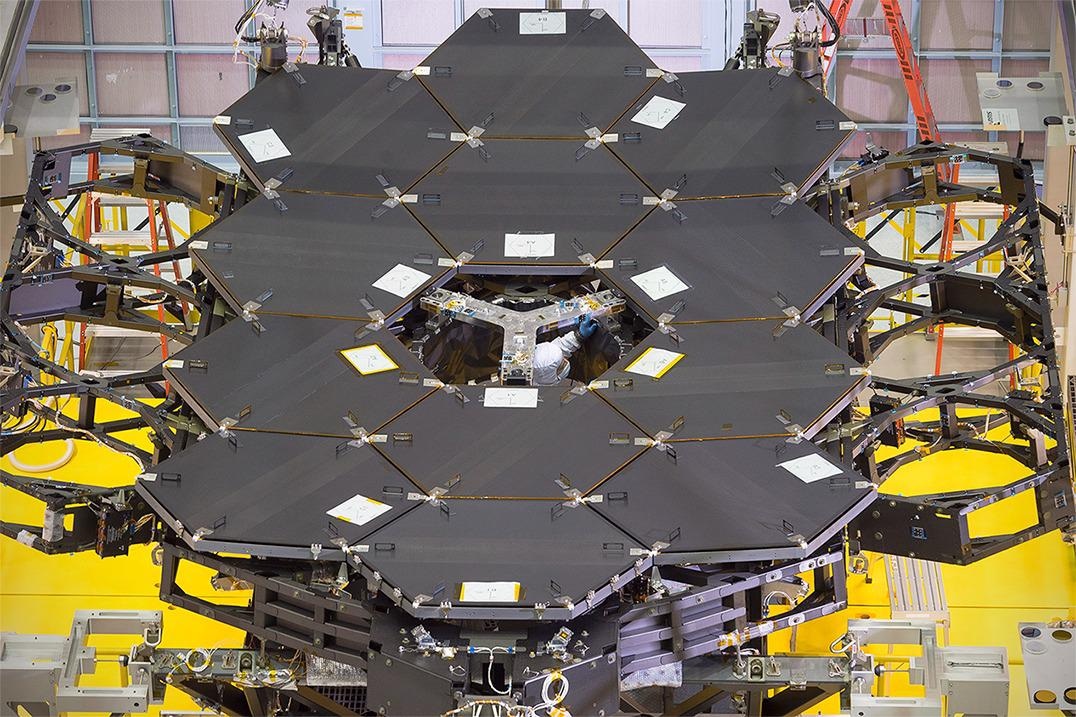
One dozen (out of 18) flight mirror segments that make up the primary mirror on NASA's James Webb Space Telescope were installed at NASA's Goddard Space Flight Center. Credits: NASA/Chris Gunn More
Will the James Webb Space Telescope come to replace the Hubble Space Telescope? How does it differ?
JWST isn’t exactly a replacement for the Hubble Space Telescope (HST), as the two are optimized for different kinds of observations (and HST is still operating!). Nevertheless, JWST can be thought of as a `successor’ to HST for its status as NASA’s flagship space-based observatory (in collaboration with the European and Canadian Space Agencies).
Astronomers expect that JWST will push the frontiers of knowledge about the cosmos with a force proportional to HST’s. Here it is worth considering HST’s towering legacy. Over three decades of service, HST has let us witness the birth of stars, discovered supermassive black holes lurking at the centers of giant galaxies, measured not only the precise rate at which the Universe is expanding, but also the rate at which that expansion is accelerating due to dark energy (a discovery that earned the 2011 Nobel Prize in Physics), and peered back in time more than 12 billion years, letting us watch the Universe evolve before our eyes.
And JWST is 100 times more powerful….
What are some of the primary research focuses that the James Webb Space Telescope will inform?
The first year of JWST observations will execute more than 300 individual projects that were selected by panels of experts from nearly 1200 proposals submitted by researchers all over the world. The approved programs will study everything from individual comets within our solar system to the large-scale structure of the Universe. In terms of primary research focus, JWST’s capabilities are optimal for studying the earliest stars and galaxies and seeing how they evolve across cosmic history.
It will also be able to see details of how stars and their planetary systems form.
Finally, JWST will be able to measure the atmospheric composition of planets both within and external to our own solar system, with sensitivity to detect molecules that could indicate habitability or perhaps even biological activity. In any case, history teaches us that the most important discoveries will come in the form of surprises that we cannot yet anticipate.
What techniques will the JWST use to observe the atmospheres of planets outside of our solar system?
This isn’t my particular line of research, but it is certainly an area where JWST is designed to have a major impact. My understanding is that JWST will acquire spectra from stars that host planets whose orbits carry them directly in front of their star. At the onset of these ‘transits’, light received from the host star will pass through the planetary atmosphere, where atmospheric molecules will absorb starlight at specific wavelengths that depend on what molecules are present.
JWST will measure the amount of light received as a function of wavelength (a ‘spectrum’), so by comparing spectra acquired during and after transit (i.e., with and without passing through the planet’s atmosphere), astronomers will be able to determine what wavelengths are absorbed by the planet’s atmosphere, and hence what specific atmospheric molecules are responsible for the absorption. This is another investigation made possible by JWST’s sensitivity to infrared light, as it is in the infrared where molecular gasses are efficient absorbers.
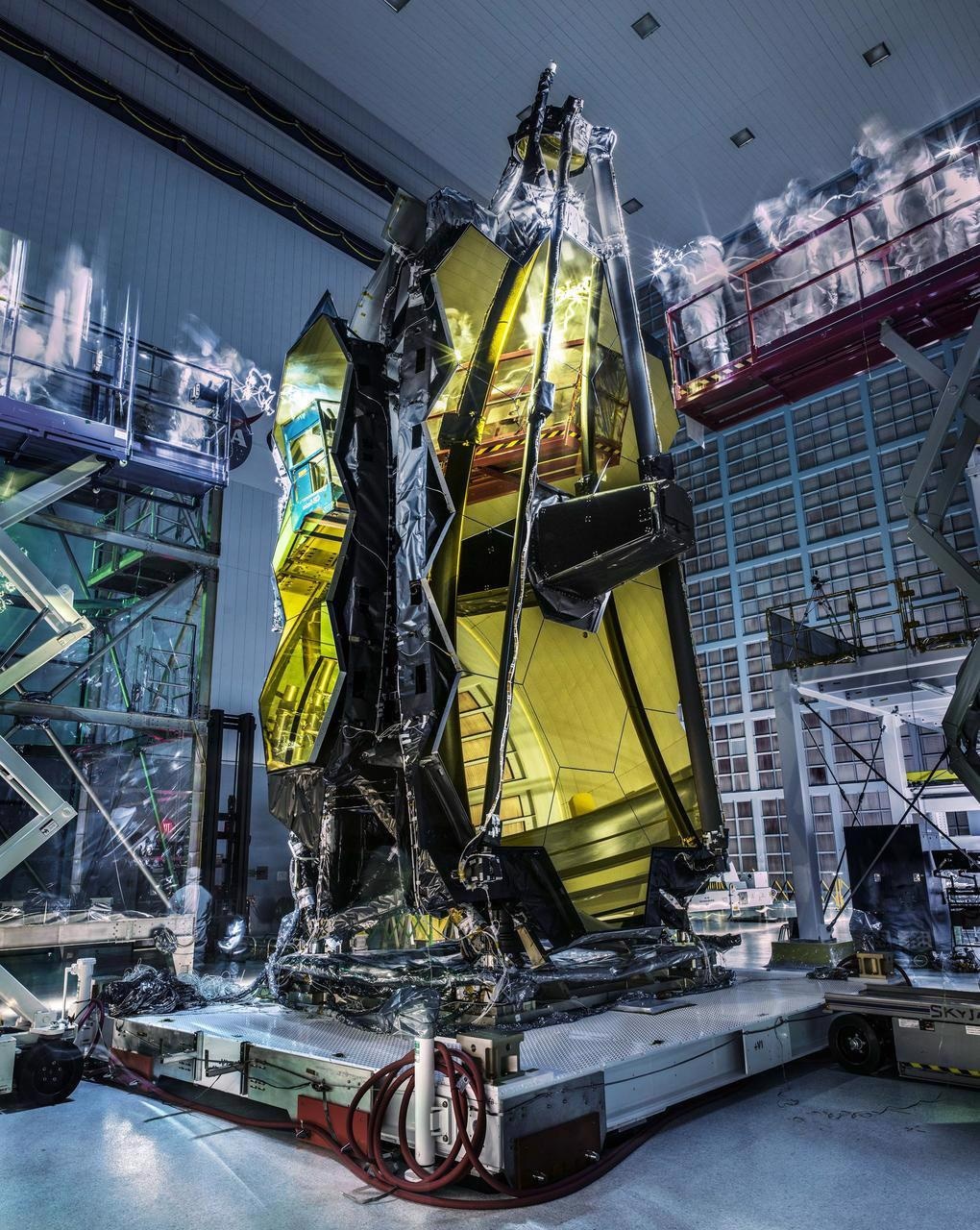
Image Credit: NASA/Chris Gunn
You have previously stated that you hope to use the James Webb Space Telescope to advance research into the detection of dark matter. Why is dark matter so difficult to detect?
I should be precise here about what I mean by ‘dark matter’, which has everything to do with how astronomers apply the laws of gravity to infer the masses of objects like galaxies.
If we use a telescope to measure a galaxy’s size and the motions of its stars, then we can plug those quantities into Newton’s law of gravity and solve for the galaxy’s mass; let’s call the mass determined in this way the galaxy’s ‘dynamical mass’.
Alternatively, we can measure the deflection of light rays passing near the galaxy, plug into the equations of Einstein’s general theory of relativity, and solve for the galaxy’s ‘lensing mass’. In principle, the dynamical and lensing masses are quantifying the same thing: the total amount of material within the galaxy, regardless of the form that the material takes (stars, gas, dust, black holes, exotic particles, etc.). And, in general, astronomers find good agreement between dynamical and lensing masses.
However, both measurements tend to be significantly larger (by factors of 10 or more) than the ‘luminous mass’, the sum of the masses of objects within the galaxy that emit, absorb, or reflect light (even after accounting for those that are too faint to detect). That gives me a working definition of ‘dark matter’: the positive quantity that is left over after subtracting the luminous mass from the amount of mass that is inferred by appealing to gravitational laws.
So defined, dark matter is astonishingly easy to detect, as we find this discrepancy between luminous and dynamical (and/or lensing) masses in nearly all galaxies. The hard part is the interpretation. Broadly speaking, the dark matter phenomenon requires that at least one of the following be true: 1) there exists some invisible substance that accounts for the deficit, or 2) the gravitational laws that we have used to infer the dynamical and/or lensing masses (general relativity and its Newtonian approximation) are somehow inadequate.
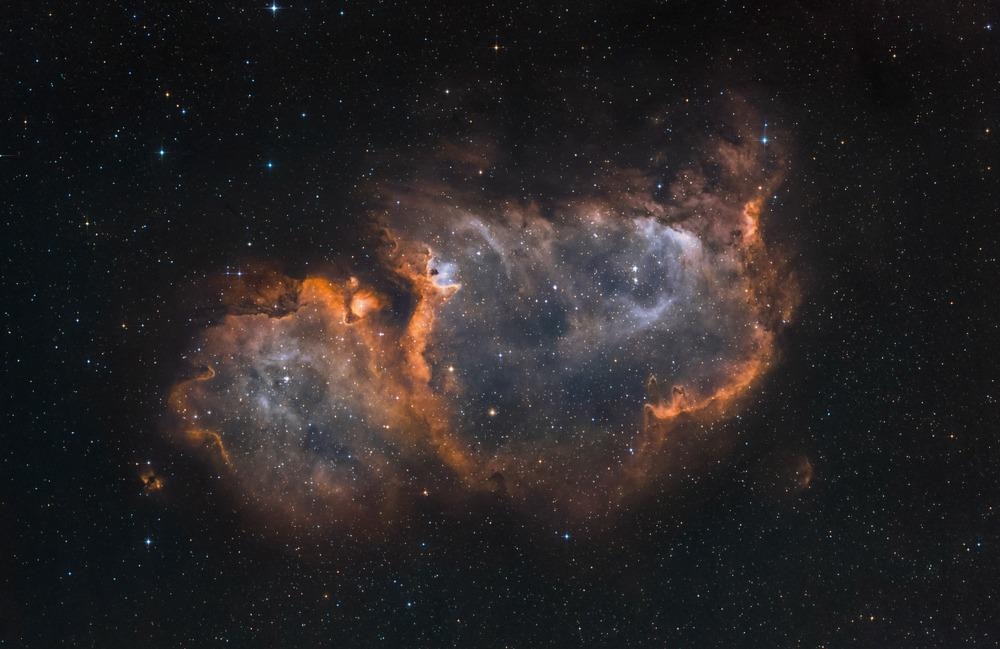
Image Credit: Marcel Drechsler/Shutterstock.com
Both options would imply exciting ‘new physics’. If dark matter is a substance, then it is presumably made of elementary particles (although there remains some possibility that it consists of ancient black holes that formed in the early Universe). But one thing that we do know quite well about dark matter is that it cannot be made of the known particles that comprise ‘normal’ matter (e.g., protons, neutrons, and electrons, or the atoms made from them that appear in the periodic table of elements); there simply aren’t enough to give the required amount of mass (see endnote*).
On the other hand, if ‘dark matter’ isn’t really matter at all, but a signal that we need a better theory of gravity, then our theorists face the formidable task of improving upon Einstein’s general theory of relativity, which has otherwise passed every available observational test.
The reason that this basic dilemma remains unsolved is that any dark matter particle has proven itself to be quite elusive. The detection of a dark matter particle would require evidence of its interaction via forces other than gravity — e.g., nuclear, electromagnetic, or even as-yet-undiscovered forces.
Yet, decades of laboratory experiments designed to detect dark matter particles interacting via non-gravitational forces have yet to produce convincing positive results. This painstaking work has taught us plenty about what dark matter is not, but has not yet revealed what it is. We need to keep looking everywhere we can, but the unsettling truth is that nature has no obligation to make all of its fundamental particles detectable by humans.
To me, that gives the dark matter problem an aspect that is somewhat philosophical: to what extent would it be useful to invoke a particle that cannot be detected?
What are dark matter halos and how can ‘wide binary stars’ help to locate them?
Dark matter ‘halos’ are the approximately spherical blobs of dark matter that we expect to form and grow as the result of dark matter particles’ mutual gravitational attraction. In the ‘substance’ interpretation of the dark matter problem, all galaxies are embedded within dark matter halos that extend far beyond the galaxies’ luminous material, ultimately providing the gravitational field that is necessary to hold individual galaxies together.
Our own Milky Way galaxy is thought to reside within a dark matter halo that has a total mass equivalent to one trillion Suns.
Even if we are not yet certain that dark matter is made of particles, astronomers can distinguish among competing dark matter particle candidates based on what their particle attributes — e.g., mass, charge, spin, proclivity for interaction via non-gravitational forces — would imply for the abundance, structure, and evolution of dark matter halos. We can even rule out some particle candidates because they would form halos that differ from the ones inferred from observations.
I am specifically interested in testing the hypothesis that dark matter is `cold’ — that is, it consists of massive particles that move slowly and interact primarily via the gravitational force. These attributes imply that ‘cold dark matter’ can be confined within relatively small halos having masses similar to the Earth’s.
In contrast, ‘warm’ dark matter, made of lighter, faster-moving particles, would quickly evaporate from low-mass halos, effectively setting a minimum halo mass at a value similar to the masses of the smallest ‘dwarf’ galaxies (approximately 10 million Suns or more).
‘Hot’ dark matter is already ruled out, because its even-lighter, even-faster particles would imply a minimum halo mass that is much larger than the masses inferred for dwarf galaxies.
For practical purposes, cold dark matter is the simplest dark matter model that we have, and so gives us a useful working hypothesis. Powerful computers can track the formation and growth of cold dark matter halos, via ‘N-body’ simulations that calculate gravitational forces between all pairs of particles and update particle trajectories accordingly.
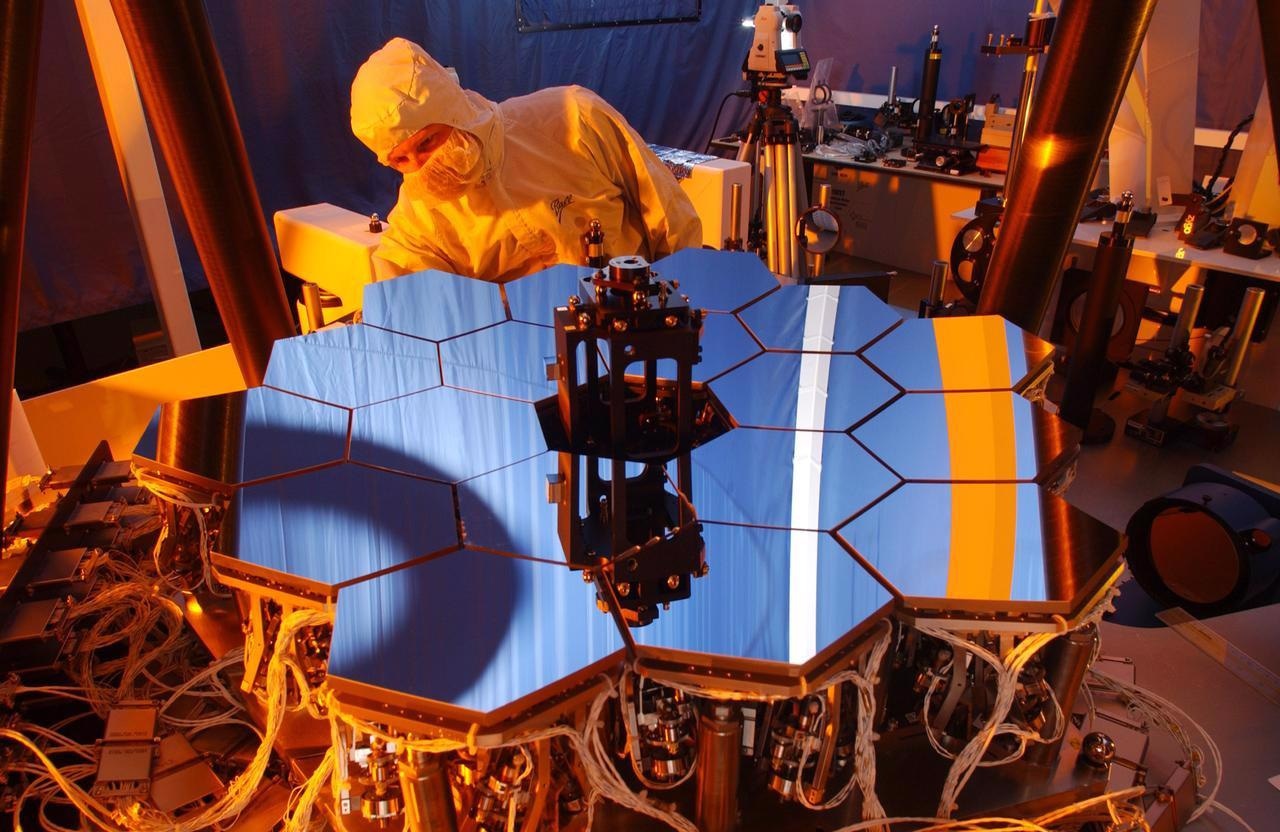
Image Credit: NASA/Chris Gunn
From these simulations a clear picture has emerged: the smallest cold dark matter halos are the first to form, with larger halos then gradually built by mergers and accretion of lower-mass halos. In this scenario, a large galaxy like the Milky Way is assembled from mergers and accretion of ancient dwarf galaxies that originally formed within their own (lower-mass) dark matter halos, which themselves formed from mergers/accretion of even lower-mass halos, and so on.
Some dwarf galaxies survive this process and orbit today as observable satellites of the Milky Way. The upshot is that throughout the halos of all galaxies, there remain countless remnants of the very first structures — the smallest halos that, owing to their low masses, never attracted sufficient gas to host stars or galaxies.
Recalling that such low-mass halos quickly evaporate if the dark matter is sufficiently ‘warm’, the existence of these completely dark, ‘sub-galactic’ dark matter halos is a distinct prediction of cold dark matter particle models.
To put it simply: If the dark matter is cold, then the halos that host all galaxies should be clumpy, full of low-mass, completely dark, sub-galactic halos left over from the earliest epoch of structure formation.
Of course, this prediction is extremely difficult to test observationally, as the clumps are made entirely of dark matter and thus are completely invisible. Nevertheless, there are a few ways we might be able to detect their influence on visible structure.
That is the ultimate goal of my James Webb Space Telescope program: to search the old stellar populations of dwarf galaxies for wide binary star systems, pairs of stars that are so weakly bound together by their mutual gravitation that they should not survive close encounters with sub-galactic cold dark matter halos.
Binary star systems are quite common, consisting of two stars orbiting a common center of mass (the point of balance between the two). Their importance to astrophysics is difficult to overstate; not only do they allow us to deduce the masses of stars, but they are also sources of phenomena like supernova explosions and gravitational waves. Since binary systems are held together only by gravity, they can be perturbed or even torn apart by the gravitational forces exerted by external objects — e.g. neighboring stars or, more relevant for my purposes, small clumps of cold dark matter.
The fragility of a binary system increases in proportion to the distance between its two constituent stars. So-called `wide’ binaries exhibit the largest separations, more than 1000 times the Sun/Earth distance, up to about one light-year (200,000 times the Sun/Earth distance). At this separation, a relatively weak gravitational tug on either star would be enough to dissociate the pair, sending each star on its own way. We do in fact observe such wide binaries within the Milky Way, where the inferred density of dark matter happens to be small enough that we can expect relatively few disruption events.
However, the dark matter halos inferred for dwarf galaxies are ten times denser than that of the Milky Way. If the dark matter is cold, then dwarf galaxies should be teeming with clumps of sub-galactic dark matter, presenting a hostile environment for wide binary stars.
My collaborator, Prof. Jorge Penarrubia (University of Edinburgh), uses computers to simulate the disruption of wide binary star systems due to repeated encounters with the many sub-galactic halos (dark clumps) within dwarf galaxies. Due to their gravitational interaction, a passing dark clump can exchange energy with the binary star system, providing the small tug necessary to separate the pair permanently. This process is so common and so efficient within dwarf galaxies that, if the dark matter is indeed cold, we expect wide binaries within these systems to be virtually non-existent today.
The catch is that we don’t actually know if wide binaries ever form within dwarf galaxies in the first place — until now, we haven’t had the observational capability of detecting them! So, if we look and don’t find any wide binaries, we can’t necessarily conclude that dark clumps must exist in order to have destroyed them. On the other hand, if we do find wide binaries surviving within dwarf galaxies, we can be confident that the dark clumps do not exist, thereby posing a serious challenge for the cold dark matter model.
How does the JWST aid the investigation of these phenomena?
JWST finally makes feasible the detection of wide binaries (if they exist) as close pairs of stars within dwarf galaxies. JWST helps to overcome two observational challenges, both of which are related to the large distances (tens of thousands of light years) to the dwarf galaxy hosts, and hence to the wide binary systems themselves.
First, at these distances, the angle between the two companions in a wide binary system will be 0.00001 degrees or less as viewed on the sky. Only telescopes having primary mirrors larger than a few meters in diameter can, in principle, distinguish two sources that close together (using visible or infrared light).
Smaller telescopes will produce images in which the pair is `blurred’ into what appears as a single point source, preventing their detection as a binary pair. In practice, even the largest telescopes on the ground cannot distinguish both companions within pairs separated by less than 0.0001 degrees, due to the blurring effects of the Earth’s atmosphere. JWST solves this problem because it has a large-diameter mirror (6.5 m) and observes from space, where there is no atmosphere to degrade image quality.
The second observational challenge is that, at the distances of dwarf galaxies, most stars are too faint to detect even with the Hubble Space Telescope.
Due to its larger light-gathering mirror, JWST will detect stars that are approximately 10-100 times fainter than what HST sees, delivering much larger samples to search for wide binary pairs.
Thus, it is the unprecedented combination of image quality and sensitivity to faint sources — both attributes derived from the fact that JWST is a large telescope in space — that gives JWST unique power to detect wide binaries.
Will you personally be using the James Webb Space Telescope to consider any other research problems?
As of now, I am not involved with other approved JWST programs. I do expect to request observations in response to future calls for proposals. If all goes well with our search for wide binary stars, we will have good reason to extend the search to other galaxies.
The telescope was launched on the 25th December 2021. Has data already been transmitted from the telescope?
The launch finally occurred early on December 25, 2021, but we don’t expect to receive the first scientific data for several more months. The spacecraft spent its first month traveling to reach its planned orbit, during which time it successfully deployed the solar shield, unfolded, and assembled the mirror array.
Over the next several weeks, the telescope will continue to cool down to its operating temperature of approximately -225 degrees Celsius. After that, the next five months will be used for aligning the mirror segments and calibrating the various detectors and instruments. If all goes well, we expect scientific operations to begin in mid-2022. For further details, I refer the reader to NASA’s excellent web page on JWST activities: https://www.jwst.nasa.gov.
How long will the telescope aim to remain in space and transmit data?
The JWST mission was designed to have a lifetime of 5-10 years. The basic limitation is the finite supply of propellant required to keep the telescope in its orbit.
One of the many pieces of good news to come over the past few weeks is that the launch and subsequent flight corrections were executed so precisely that there may be enough fuel remaining on board to maintain JWST’s orbit for 20 years! Any extension beyond that limit would require a robotic servicing mission, but (to my knowledge) there are no plans or even capability for that right now.
How significant will the James Webb Space Telescope be to the progression of research into our own solar system and those beyond it?
This is one of many areas where JWST will be a game-changer. Within our solar system, JWST will observe Mars and the outer planets, their moons, asteroids, comets, and the icy bodies that comprise the outer solar system.
With its infrared sensitivity and ability to measure fine features in the intensity of light as a function of wavelength, JWST will be able to discern the molecular composition of planetary atmospheres. With observations of Mars, for example, JWST can search for organic molecules associated with past or present life. Observing extra-solar planets, those same capabilities can search for indications of habitability, one of the fundamental goals of exo-planetary studies.
JWST will also have exquisite capabilities for shielding the light from bright stars in order to observe their relatively faint planets directly. Finally, by observing the dusty disks around proto-stars, JWST will see directly the processes that determine the formation of stars and their planetary systems.
What is personally the most exciting aspect of the launch of this telescope for you?
I entered graduate school in the fall of 2000, and I have been familiar with the JWST project for my entire career in astronomy. For most of that time, it has seemed a distant aspiration, so it is both impressive and surreal to see JWST finally launched and on track for success.
But my strongest reaction is sentimental. Like many people, for the past several years I’ve been waking up most days hesitant to open the newspaper, weary of being confronted with the latest deterioration in the world.
JWST reminds us that humanity is still capable of greatness, that we can decide it is worth the time, effort, and money simply to see what’s out there, that in contemplating the Universe we can all share a deep connection not just with each other but also with our ancestors going back to the cave painters.
The public’s outpouring of excitement, support, and good wishes surrounding the launch tells me that most people feel the same way. That has been gratifying and validating, and heightens my sense of responsibility to help the mission succeed scientifically.
EndNote*
The number of these ‘normal’ particles is limited by:
1) the observed prevalence of deuterium, an isotope of hydrogen that was produced via nuclear fusion during the Universe’s first few seconds
2) observations of the degree to which these ‘normal’ kinds of particles were able to cluster together during the subsequent few-hundred-thousand years.
Where can readers find more information?
https://jwst.nasa.gov/
About Dr. Matthew Walker
Dr. Walker is currently an Associate Professor of Physics at Carnegie Mellon University, where he has served on the faculty since 2013. Before that, he completed his Ph.D. in Astronomy & Astrophysics at the University of Michigan in 2007. Between graduate school and CMU, he did three years of postdoctoral research at the Institute of Astronomy at the University of Cambridge (2007 - 2010), and another three as a Hubble Postdoctoral Fellow at Harvard University (2010 - 2013).
Disclaimer: The views expressed here are those of the interviewee and do not necessarily represent the views of AZoM.com Limited (T/A) AZoNetwork, the owner and operator of this website. This disclaimer forms part of the Terms and Conditions of use of this website.