By Ankit SinghReviewed by Susha Cheriyedath, M.Sc.May 8 2024
Superconductivity, the phenomenon where certain materials exhibit perfect electrical conductivity below a critical temperature, has intrigued scientists and engineers for over a century due to its transformative potential in various fields such as power transmission, computing, and medical imaging like magnetic resonance imaging (MRI).
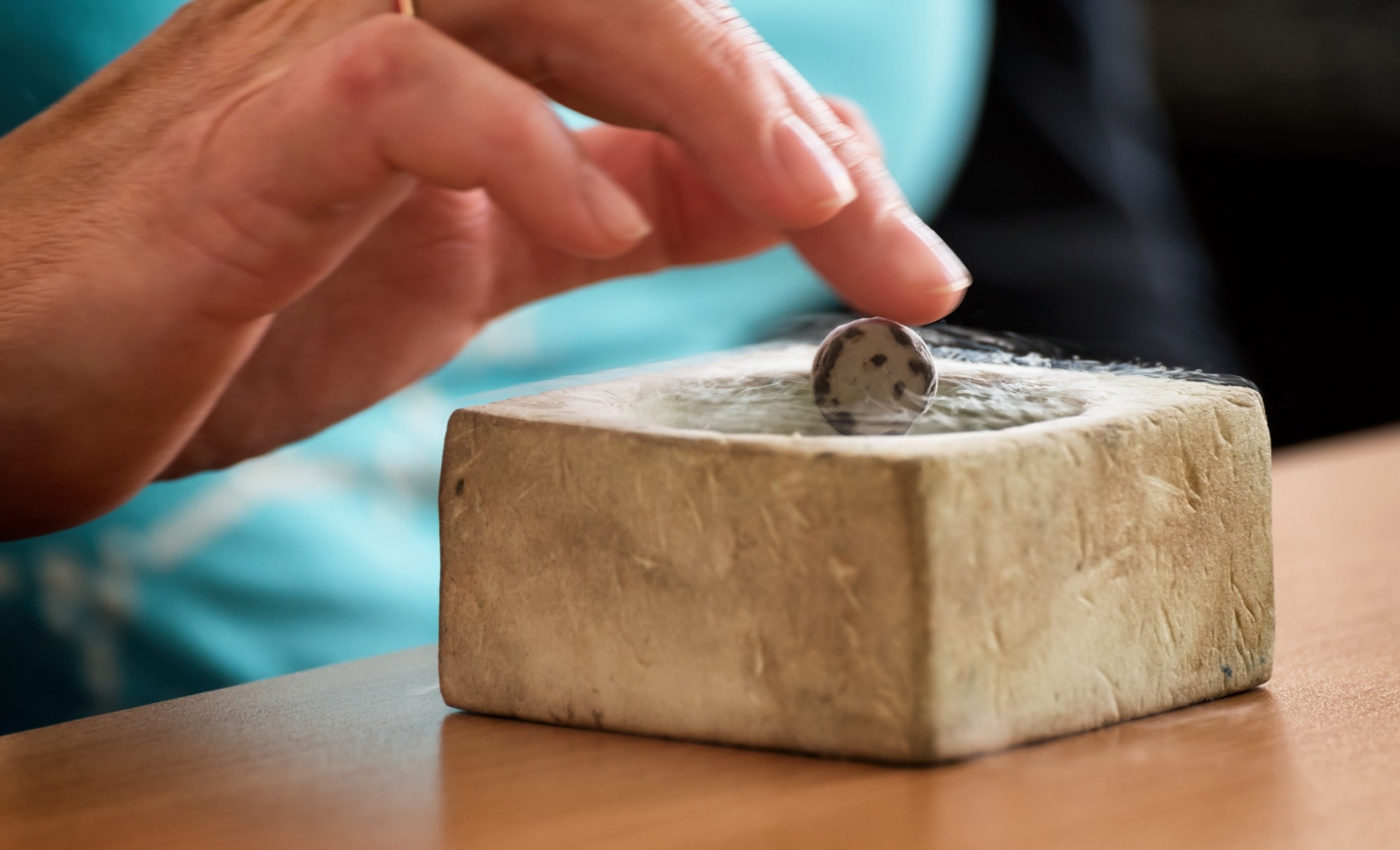
Image Credit: Forance/Shutterstock.com
However, conventional superconductors often require extremely low temperatures to maintain their superconducting state, limiting their widespread use. To overcome this limitation, researchers have turned to one-dimensional systems, which offer unique advantages in advancing superconductivity.
This article delves into the principles of superconductivity in one-dimensional systems. It also discusses the diverse applications, current challenges, and promising advancements in the field.
Chilling Century of Superconductors
Superconductivity, first discovered by Heike Kamerlingh Onnes in 1911, has evolved significantly over the years. Initially observed in materials cooled to extremely low temperatures, understanding of the phenomenon deepened with the elucidation of mechanisms like Cooper pair formation. The discovery of high-temperature superconductors (HTS) in the late 1980s marked a breakthrough, pushing critical temperatures beyond traditional limits, but these materials still required cooling with liquid nitrogen.1
In recent decades, research has shifted towards one-dimensional (1D) systems. In contrast to traditional three-dimensional (3D) superconductors, where electrons pair up across the entire material, 1D superconductors confine electrons to move along a single direction, leading to potentially higher critical temperatures (Tc). This shift represents a pivotal moment in the evolution of superconductivity research as scientists seek to harness the unique properties of one-dimensional systems for groundbreaking advancements in various fields.2
Unveiling the Core Principles: Cooper Pairs and BCS Theory
The Bardeen-Cooper-Schrieffer (BCS) theory stands as the leading explanation for conventional superconductivity. According to this theory, electrons join forces to form Cooper pairs, cohesive electron states facilitated by interactions with lattice vibrations, known as phonons. As these pairs navigate the material, they effectively sidestep collisions with imperfections, resulting in the material's ability to conduct electricity without resistance. The critical temperature (Tc) at which superconductivity manifests is dictated by the intensity of the electron-phonon interaction.1,2
However, the BCS theory faces theoretical challenges when it comes to 1D systems. In a strictly 1D system, phonons with a wavevector matching twice the electron momentum for Cooper pair formation are absent. Nevertheless, recent research indicates that alternative pairing mechanisms can arise in 1D materials with specific characteristics.2
Beyond Phonons: New Pairing Mechanisms
Some of the new pairing mechanisms being explored for 1D superconductivity include:
- Electron-Electron Repulsion: In certain 1D materials, strong repulsive interactions between electrons can lead to superconductivity. When the confinement of electrons in 1D limits their available states, these repulsive interactions can force electrons to occupy opposite momentum states, effectively pairing them up. This mechanism, known as "spin-fluctuation-mediated" superconductivity, is being investigated in materials with strong electron correlations.2
- Excitonic Pairing: In some 1D materials, interactions between electrons and holes can lead to the formation of bound excitonic states. These excitons can condense at low temperatures, giving rise to superconductivity through a mechanism distinct from phonon-mediated pairing.2
- Plasmon-Mediated Pairing: Collective oscillations of electrons in a 1D system can create plasmons, which are quasiparticles that behave like light. These plasmons can mediate attractive interactions between electrons, potentially leading to superconductivity.2
By investigating these alternative pairing mechanisms, scientists are broadening their understanding of how superconductivity can emerge in 1D systems.
Advantages of 1D Superconductors
Despite the theoretical hurdles, 1D superconductors offer several potential advantages over their 3D counterparts. One advantage is that the confined geometry of 1D materials allows for stronger electron-electron interactions, which could lead to better pairing at higher temperatures. Additionally, 1D materials often have "flat bands" in their electronic structure, where electrons have similar energies. These flat bands can facilitate Cooper pair formation and potentially increase Tc.2
Recent advances in atomistic manipulation techniques have made it possible to create designer 1D materials with customized properties. For example, researchers have successfully synthesized atomic chains with precisely controlled atomic arrangements and chemical compositions. This development paves the way for fine-tuning electron interactions and exploring unconventional pairing mechanisms.3
Emerging Applications and Technological Implications
While achieving perfect electrical conductivity remains a primary goal, 1D superconductors hold promise for various technological applications even at lower Tc values. Their unique electronic properties make them ideal candidates for studying fundamental physical phenomena like Majorana fermions and hypothetical particles with potential applications in topological quantum computing.
Majorana fermions are quasiparticles that exhibit non-abelian braiding statistics, a highly desirable property for fault-tolerant topological quantum computing. Recent studies suggest that 1D superconductors with strong spin-orbit coupling might host Majorana fermions, offering a path toward building a new generation of quantum computers with unprecedented processing power.4,5
Additionally, 1D superconductors can be integrated with other materials to create "designer" heterostructures with tailored functionalities. For example, 1D superconductors integrated with ferromagnetic materials can create "s-wave/ferromagnet" heterostructures with interesting spintronic properties.
The proximity effect can induce superconductivity in the ferromagnetic layer, leading to systems where spin currents can be manipulated with superconducting efficiency. This could enable the development of ultra-low power spintronic devices with faster operation speeds.6
Moreover, single-electron transistors (SETs) based on 1D superconductors are emerging that could revolutionize nanoelectronics by offering superior sensitivity and low power consumption. Their ability to detect the presence of a single electron makes them ideal for applications in biosensing and quantum information processing.7
1D superconductors also have potential applications in energy storage. While conventional superconductors require cryogenic temperatures for efficient storage, certain 1D materials exhibit superconductivity at temperatures closer to liquid hydrogen levels, which are more achievable and economical than liquid nitrogen. Developing high-performance 1D superconductors suitable for energy storage could revolutionize grid management and enable large-scale integration of renewable energy sources.8
Finally, the unique electrical properties of 1D superconductors make them ideal candidates for developing high-sensitivity nanoprobes. These nanoprobes could be used for ultrafast scanning tunneling microscopy (STM) and scanning electron microscopy (SEM), providing unprecedented resolution for probing the electronic structure of materials at the atomic level. Additionally, 1D superconducting nanoprobes could be employed in biomedical applications for high-resolution imaging of biological molecules and cells.9
Challenges and Opportunities
Despite the exciting potential, research on 1D superconductivity faces several challenges. Firstly, it is difficult to fabricate high-quality 1D materials with well-defined properties, as common synthesis techniques often produce impurities and defects that can affect superconductivity. Additionally, advanced experimental techniques are required to study the superconducting properties of these nanoscale materials.2
Furthermore, the theoretical understanding of superconductivity in 1D systems is still developing. While scientists are exploring alternative pairing mechanisms beyond traditional phonons, a unified theoretical framework for describing superconductivity in 1D is yet to be established.2
Recent Breakthroughs
Recent studies have made significant strides in advancing one-dimensional superconductivity. Researchers have demonstrated the fabrication of ultrathin nanowires with enhanced superconducting properties, achieving record-high critical temperatures.
For example, a study published in Superconductor Science and Technology reported the synthesis of ultrathin niobium nanowires exhibiting robust superconductivity up to temperatures close to 10 Kelvin, surpassing previous records for one-dimensional superconductors.10
In a recent study, researchers at the University of Manchester achieved superconductivity in a newly created 1D system at relatively high magnetic fields. This development demonstrates the potential for achieving robust superconductivity in 1D materials and paves the way for exploring superconductivity in the quantum Hall regime, a long-standing challenge in condensed matter physics.11
Moreover, advancements in materials engineering have led to the discovery of novel one-dimensional superconducting materials, such as twisted bilayer graphene. Researchers have shown that by precisely twisting two layers of graphene at a specific angle, exotic superconducting states emerge, offering new avenues for exploring unconventional superconductivity in low-dimensional systems.12
Future Prospects and Conclusion
The future of one-dimensional superconductivity holds tremendous promise for both fundamental research and practical applications. Continued advancements in nanofabrication techniques will enable the precise control of one-dimensional structures, pushing the boundaries of superconducting performance to even higher temperatures and critical current densities. By combining advanced theory and experimentation, scientists can unravel the unique physics governing superconductivity in 1D systems.
Moreover, interdisciplinary collaborations between physicists, material scientists, and engineers will drive innovation in novel materials and device architectures, unlocking new functionalities and their potential for transformative technological applications.
In conclusion, 1D superconductors are at the forefront of the search for high-temperature superconductivity and quantum technologies. With continuous research and technological advancements, these systems have the potential to unlock new horizons in both fundamental physics and practical applications, leading to a future driven by efficient and resilient superconducting technologies.
More from AZoQuantum: An Introduction to Quantum Computing
References and Further Reading
- Rey, C., & Malozemoff, A. (2014). Fundamentals of superconductivity. Superconductors in the Power Grid, 29-73. https://doi.org/10.1016/B978-1-78242-029-3.00002-9
- Arutyunov, K. Y., Golubev, D. S., & Zaikin, A. D. (2008). Superconductivity in one dimension. Physics Reports, 464(1-2), 1–70. https://doi.org/10.1016/j.physrep.2008.04.009
- Meng, Y., Wang, W., & Ho, J. C. (2022). One-Dimensional Atomic Chains for Ultimate-Scaled Electronics. ACS Nano. https://doi.org/10.1021/acsnano.2c06359
- Marra, P. (2022). Majorana nanowires for topological quantum computation. Journal of Applied Physics, 132(23), 231101. https://doi.org/10.1063/5.0102999
- Val'kov, V. V., Shustin, M. S., Aksenov, S. V., Zlotnikov, A. O., Fedoseev, A. D., Mitskan, V. A., & Kagan, M. Y. (2021). Topological superconductivity and Majorana states in low-dimensional systems. Physics-Uspekhi. https://doi.org/10.3367/ufne.2021.03.038950
- Mel'nikov, A. S., Mironov, S. V., Samokhvalov, A. V., & Buzdin, A. I. (2021). Superconducting spintronics: state of the art and perspectives. Physics-Uspekhi. https://doi.org/10.3367/ufne.2021.07.039020
- Katkar, A. S., Gupta, S. P., Granata, C., Nappi, C., Prellier, W., Chen, L.-J., & Walke, P. S. (2020). Advanced Room Temperature Single-Electron Transistor of a Germanium Nanochain with Two and Multitunnel Junctions. ACS Applied Electronic Materials, 2(7), 1843–1848. https://doi.org/10.1021/acsaelm.0c00242
- Dou, F.-Q., & Yang, F.-M. (2023). Superconducting transmon qubit-resonator quantum battery. Physical Review A, 107(2). https://doi.org/10.1103/physreva.107.023725
- Bian, K., Gerber, C., Heinrich, A.J. et al. Scanning probe microscopy. Nat Rev Methods Primers 1, 36 (2021). https://doi.org/10.1038/s43586-021-00033-2
- Hazra, D., Tsavdaris, N., Jebari, S., Grimm, A., Blanchet, F., Mercier, F., Blanquet, E., Chapelier, C., & Hofheinz, M. (2016). Superconducting properties of very high quality NbN thin films grown by high temperature chemical vapor deposition. Superconductor Science and Technology, 29(10), 105011. https://doi.org/10.1088/0953-2048/29/10/105011
- Barrier, J., Kim, M., Kumar, R.K. et al. One-dimensional proximity superconductivity in the quantum Hall regime. Nature 628, 741–745 (2024). https://doi.org/10.1038/s41586-024-07271-w
- Wang, ZJ., Kong, X., Huang, Y. et al. Conversion of chirality to twisting via sequential one-dimensional and two-dimensional growth of graphene spirals. Nat. Mater. 23, 331–338 (2024). https://doi.org/10.1038/s41563-023-01632-y
-
Disclaimer: The views expressed here are those of the author expressed in their private capacity and do not necessarily represent the views of AZoM.com Limited T/A AZoNetwork the owner and operator of this website. This disclaimer forms part of the Terms and conditions of use of this website.