Fundamental information related to a physical system could be revealed by studying noise processes. The information could be temperature of the system [1, 2, 3], the quantization of charge carriers [4], or the nature of many-body behavior [5]. Moreover, noise measurements are crucial in the case of applications such as sensing in which the inherent noise of a detector governs its ultimate sensitivity.
This article describes a generic method for the measurement of electronic noise in a device when the spectral density of its inherent noise is less when compared to that of the measurement apparatus. The method is implemented with the help of a pair of MFLI Lock-in Amplifiers, both of which have the Digitizer option MF-DIG.
Demonstration of the measurement is performed by measuring the noise of a Superconducting Quantum Interference Device (SQUID) magnetometer. SQUIDs are essentially run by supplying DC bias current and measuring voltage, and in general, the noise floor of the voltage preamplifier is the limiting factor in the measurement. A noise floor of 1 nV/√Hz is needed for a SQUID with a gain (transfer function) of VΦ = 1 mV/Φ0 and a target sensitivity of S = 1 μΦ0/√Hz.
Although this noise floor can be achieved using certain commercial amplifiers and dedicated SQUID readout electronics, a majority of the general purpose, wide bandwidth preamplifiers—for example, those used in the front end of lock-in amplifiers—can only achieve a few nV/√Hz. The voltage noise density for the voltage input of an MFLI Lock-in Amplifier from Zurich Instruments is illustrated in Figure 1.
.jpg)
Figure 1. The voltage noise density at the Voltage Input of an MFLI Lock-in Amplifier plotted for available input ranges [6].
At the highest gain setting, the noise floor saturates at 2.5 nV/√Hz above the value of 1 kHz. The noise increases below the value of 1 kHz and reaches 7 nV/√Hz at 10 Hz. Although these noise figures are exceptional, they would be inadequate for the characterization of the noise of several DC SQUIDs, specifically at low frequencies.
Signals below the noise floor of an amplifier can be resolved by making two independent measurements with two different amplifiers and calculating the cross-correlation of their outputs [7, 8]. In an average collected over several measurements such as these, signals uncorrelated in the two amplifiers (for example, amplifier noise) are attenuated; by contrast, correlated persistent signals are preserved.
Two nominally identical measurement channels with the potential to perform real-time sampling of data simultaneously are needed to carry out such a cross-correlation measurement. A number of data acquisition devices (DAQs) do not have the ability to capture data simultaneously; moreover, when the data is captured from more than one channel at a time, interleaving of samples occurs and the sampling rate is divided by the number of channels.
DAQs with the ability to perform simultaneous sampling are very costly in general. The MFLI Digitizer option can be used to capture nearly 2.5 M samples per channel, with 16-bit resolution, at rates of up to 60 MHz. Synchronization of the two MFLIs enables this data to be captured truly simultaneously with the help of the Zurich Instruments multi-device synchronization (MDS) offered by the LabOne® software.
The low-noise floor of the MFLI is a good starting point for the measurement. The MFLI is a multi-functional device and its Auxiliary Outputs can also be used to control all other parameters of the SQUID measurement. As illustrated in Figure 2, in this experiment, a four-terminal configuration has been used to measure a DC SQUID.
.jpg)
Figure 2. Top: cabling for automatic synchronization of two MFLI instruments using the multi-device synchronization (MDS) tool. Bottom: wiring schematic for noise measurements. The AC and DC voltages from the right MFLI are used to control the output of a low-noise, precision current source. There are two flux bias lines on the chip inductively coupled to the SQUID loop. An AC flux is applied using the Signal Output of the left MFLI and a DC flux using the Auxiliary Output of the right MFLI.
The SQUID is somewhat unusual because it has graphene Josephson junctions [9, 10, 11]. The normal-state resistance of the junctions is of the order 50 Ω and the SQUID has a maximum gain of about 400 μV/Φ0. An Oxford Instruments Triton 400 Cryofree dilution refrigerator is used to cool the device to a temperature less than 10 mK.
A low-noise current source (manufactured at Lancaster University) is used to current-bias the SQUID, where the current source takes an AC voltage VAC from an MFLI Signal Output to set the AC current, and a DC voltage VDC from an MFLI Auxiliary Output to set the DC current. The source supplies a current of (10 μA/V)VDC + (10 nA/V)VAC in this measurement.
Two flux bias lines exist on the device chip and can produce a local magnetic field in the SQUID loop. One of the flux bias lines is used to produce a DC flux by connecting it to an Auxiliary Output of an MFLI through a 10-kΩ resistor. The other line offers an AC flux when it is connected to the Signal Output of the second MFLI through a 10-kΩ resistor.
This configuration can be used to simultaneously measure the differential conductance as well as the SQUID's gain. The voltage measurement lines linked to the SQUID are divided at the fridge’s coldest part in a way that each channel possesses its own pair of wires inside the cryostat. Cryogenic RC filters are used to filter all the lines with a cut-off frequency of 1 MHz at a temperature of 4 K.
The Digitizer option in the MFLIs is used to capture real-time data segments and subsequently calculate the discrete Fourier transform. The data from the two devices must be captured simultaneously to carry out a cross-correlation. As illustrated in Figure 2 (Top), this is performed by connecting the two MFLIs so that each instrument makes use of the same clock and includes a common trigger for the synchronization of the time stamps of both devices by MDS.
Then, the Scope module is set on each MFLI to capture data by means of the common trigger. In reality, a small, random time offset exists between the two Scope traces during initialization. This time interval is read out at the beginning of each acquisition and the Scopes are restarted if the interval falls outside a given threshold. In case the initial offset is less than the threshold, it will remain so for the duration of the data capture.
Cross-Correlation Measurements
The result of each measurement is a time series of voltages V1(t) from the first MFLI and another series V2(t) captured simultaneously by the second MFLI. Each signal has a common component Vs(t), which is the signal that needs to be measured, and an independent noise component Vni(t):
V1(t) = Vs(t) + Vn1(t), V2(t) = Vs(t) + Vn2(t) |
(1) |
The cross-correlation spectrum S(f) is computed as the dot product of the complex conjugate of the Fourier transform of one signal with the Fourier transform of the other:
S(f) = F[V1(t)]*⋅F[V2(t)] |
(2) |
The result is averaged over several repeated measurements:
.jpg) |
(3) |
In the case of totally uncorrelated noise, the noise floor is attenuated by a factor of 10 (20 dB) and any correlated signals are preserved upon averaging over 104 measurements.
Results and Outlook
The results of characterization of Zurich Instruments’ DC SQUID with the help of the cross-correlation method are illustrated in Figure 3. In Figure 3(b), a cross-correlation spectrum averaged over 105 measurements has been compared with the spectrum from a single MFLI with the same number of averages.
.jpg)
Figure 3. (a) IV characteristic (black) of the SQUID when biased at 0.5 Φ0, minimum gain, and average noise around 62 Hz (red) taken at different points along the IV curve. (b) Noise spectrum from a single channel with 105 averages and noise spectrum after correlating two channels with 105 averages (black). (c) Correlated noise power spectral density around 62, 323, and 675 kHz as a function of the number of averages. The noise floor of the correlated measurement is around 0.5 nV/√Hz. (d) Correlated noise spectra after 1, 103, and 105 averages. The curves have been smoothed for clarity. The blue, red, and black-shaded areas show the regions used for panel c. Note: For all spectra, peaks at 50 Hz (mains electric frequency) and the higher harmonics have been removed for clarity.
A more precise spectrum of a single channel noise can be obtained by averaging a single channel; by contrast, uncorrelated noise signals are attenuated by the average of the cross-correlation to reveal the spectrum of signals correlated between the two channels. The noise floor in the cross-correlation is considerably lower over the entire frequency range and reaches approximately ∼0.5 nV/√Hz above a few tens of Hertz.
Figures 3(c) and 3(d) illustrate the variation of the noise in the cross-correlation with the number of averages. The decrease in the noise floor at three frequencies of interest is shown in Figure 3(c). The complete spectrum for different numbers of averages is shown in Figure 3(d). The spectrum becomes flatter with more averages, indicating that the 1/f component of the uncorrelated noise is larger compared to the correlated (inherent) noise.
This is evident from Figure 3(d) where the reduction in the noise at 1 kHz is very little from 103 to 105 averages but decreases continuously at about 10 Hz. In the same way, this is evident in Figure 3(c) where the noise at 62 Hz seems to decrease more when compared to the noise at 323 and 675 Hz.
The SQUID was fully superconducting and the bias current was set to zero to achieve the results illustrated in Figures 3(b) to 3(d). It is expected that the SQUID’s inherent noise will be extremely low in this state and hence the results exhibit the noise floor of the measurement setup. From Figure 3(c), it can be seen that the noise floor saturates at ∼0.5 nV/√Hz after ≈3 x 103 averages. From this, it can be concluded that an inherent noise exists in the device or the measurement setup of ∼0.5 nV/√Hz.
The circuit’s noisiest part is expected to be the cryogenic filters in the current bias connections, which are 200 Ω each and will produce a Johnson noise of 0.3 nV/√Hz overall. Although this magnitude is similar to that of the measured noise floor, it is very low to account for all of the correlated noise. There could be additional noise from other parts of the measurement circuit, and the 200-Ω resistors might not be fully thermalized to the fridge’s 4 K stage.
Once the noise of the measurement setup is characterized, the same method can now be used to analyze the SQUID’s noise in different operating conditions. Figure 3(a) illustrates the IV characteristic of the SQUID upon being biased at a point of minimum flux sensitivity, together with the correlated noise values.
It must be noted that since noise is measured at relatively low frequencies, long measurement times are required for collecting a large number of averages. In order to confirm that the SQUID does not drift from its operating point, it is necessary to compare individual signal spectra during the averaging process. It has been found that it is generally possible to bias Zurich Instruments’ graphene junction SQUIDs at a given operating point and they will remain in the same condition for many days with no measurable drift.
The noise does not have measurable dependence on the magnitude of the bias current and is equal to 0.5 nV/√Hz in the superconducting region, below IC. It is observed that the noise increases with bias current above IC. In the absence of a correlated measurement, this behavior would be nearly completely invisible as the noise from the device only violates the single channel noise floor above IDC 5 μA.
The intrinsic sensitivity of Zurich Instruments’ SQUID magnetometer can be quantified by removing the influence of uncorrelated noise in the voltage measurement, enabling the study of the origin of inherent and environmental noise having an impact on the SQUID.
The example illustrated in this article shows the general use of cross-correlation measurements while studying signals that are usually hidden below the noise floor. Here, it is possible to attenuate the noise floor of the input amplifier of the MFLI by using two MFLIs in parallel.
References
[1] D. Rothfuß, A. Reiser, A. Fleischmann, and C. Enss. Noise thermometry at ultra-low temperatures. Philos. Trans. Royal Soc. A, (374), 2016.
[2] Z. Iftikhar, A. Anthore, S. Jezouin, F. D. Parmentier, Y. Jin, A. Cavanna, A. Ouerghi, U. Gennser, and F. Pierre. Primary thermometry triad at 6 mk in mesoscopic circuits. Nature Communications, 7:12908, September 2016.
[3] A. Shibahara, O. Hahtela, J. Engert, H. van der Vliet, L. V. Levitin, A. Casey, C. P. Lusher, J. Saunders, D. Drung, and Th. Schurig. Primary current-sensing noise thermometry in the millikelvin regime. Philosophical Transactions of the Royal Society of London A: Mathematical, Physical and Engineering Sciences, 374(2064), 2016.
[4] M. Dolev, M. Heiblum, V. Umansky, Ady Stern, and D. Mahalu. Observation of a quarter of an electron charge at the ν = 5/2 quantum hall state. Nature, 452(7189):829–834, April 2008.
[5] Jesse Crossno, Jing K. Shi, Ke Wang, Xiaomeng Liu, Achim Harzheim, Andrew Lucas, Subir Sachdev, Philip Kim, Takashi Taniguchi, Kenji Watanabe, Thomas A. Ohki, and Kin Chung Fong. Observation of the Dirac fluid and the breakdown of the wiedemann-franz law in graphene. Science, 351(6277):1058–1061, 2016.
[6] Zurich Instruments. MFLI User Manual, 2017.
[7] T. Chen and A. van der Ziel. Hanbury brown-twiss type circuit for measuring small noise signals. Proceedings of the IEEE, 53(4):395–395, April 1965.
[8] S. S. Wolff. On the brown-twiss circuit. Proceedings of the IEEE, 53(8):1140–1141, Aug 1965.
[9] Caglar Girit, V. Bouchiat, O. Naaman, Y. Zhang, M. F. Crommie, A. Zettl, and I. Siddiqi. Tunable graphene dc superconducting quantum interference device. Nano Letters, 9(1):198–199, 2009. PMID: 19090696.
[10] G. Nanda, J. L. Aguilera-Servin, P. Rakyta, A. Kormányos, R. Kleiner, D. Koelle, K. Watanabe, T. Taniguchi, L. M. K. Vandersypen, and S. Goswami. Current-phase relation of ballistic graphene Josephson junctions. Nano Letters, 0(0), 2017. PMID: 28474892.
[11] M. D. Thompson, M. Ben Shalom, A. K. Geim, A. J. Matthews, J. White, Z. Melhem, Yu. A. Pashkin, R. P. Haley, and J. R. Prance. Graphene-based tunable squids. Applied Physics Letters, 110(16):162602, 2017.
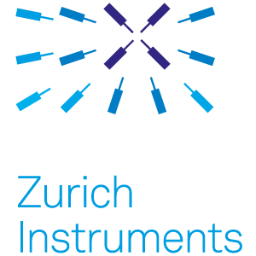
This information has been sourced, reviewed and adapted from materials provided by Zurich Instruments AG.
For more information on this source, please visit Zurich Instruments AG.